The microstructure of a material determines many of its most important properties, such as its longevity and its response to stress. However, data about metals at the microstructural level and how processing affects their structure is sparse. Most metal components for aviation are specified on the assumption of worst-case performance.
More knowledge about the microstructure of metals is required as engineers work to reduce the environmental impact of aviation through weight reductions and other improvements, they are looking at new manufacturing processes and new materials.
It is also required as metal additive manufacturing (AM) rolls out for the aerospace sector. AM has extremely short heating and cooling timescales and compared to subtractive manufacturing techniques, little is known about the affects of AM on metals. This makes estimates of the safety and durability of 3D printed parts extremely difficult.
Modeling and simulation
Software is becoming available to help assess the microstructure of metals during design and testing to improve a part’s properties and quality. Earlier this year multi-physics simulation company e-Xstream partnered with the IMDEA Materials Research Institute, in Madrid, Spain, to make IMDEA’s metal microstructure models commercially-available.
IMDEA, which is best known for its work on structural materials, employs around 120 researchers. The engineers and scientists at IMDEA are applying principles of integrated computational materials engineering (ICME) in the approach to developing the microstructural models. Professor Javier Segurado from IMDEA says, “We helped develop the latest generation of composites and now we are working on the microscopic characteristics of metals”.
“By linking materials models at different length scales we can simulate the design of parts, accounting for the material and the process. We can model what happens to the grains of the metal at the microstructural level and predict the properties of the produced part at different length scales.
“People want to understand materials better, leading to mechanical parts with improved performance. By designing the material at the microstructural level, its use can be optimized.”
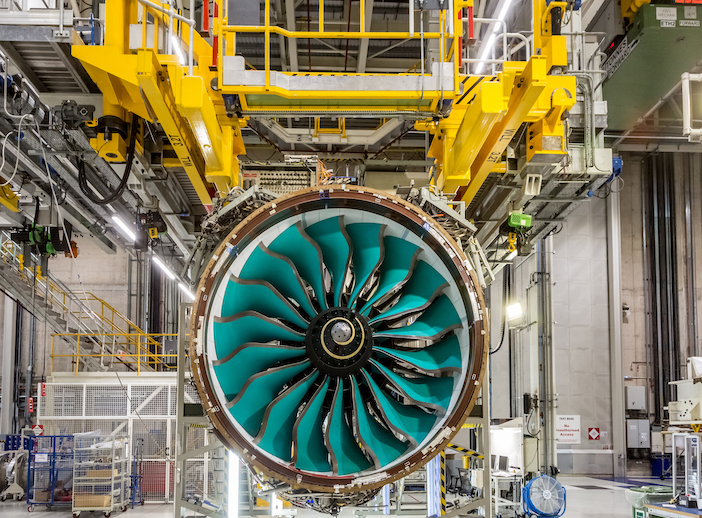
In a simplistic way, the microstructural modeling being done with metals at IMDEA is a continuation of the composites research looking at fibers and matrices within the material, “but there are big differences between the materials as well,” says Segurado. “Metals are heterogenous like composites but are not glued together. The mechanisms for deformation traverse grain boundaries. Composites have a clear structure, whereas in a metal everything is more intricate.”
Segurado says that different levels of complexity demand different levels of modeling and that both depend on the final application. “For a relatively large part, such as a disc in a jet engine, you may only have to look at strain and temperature response at a macro level. We only go smaller, down to the atomic scale if we want, when demanded by the application.
“The main purpose of the partnership with e-Xstream is to understand the micro or mesoscopic level. It allows us to consider that the metal is not monolithic anymore but heterogenous and made of different grains that have different orientations, shapes, and sizes.
“How you make the part affects the grain size, orientation and shape. The type of models we are developing accounts for this and then we can simulate the mechanical responses.”
Using metal microstructural modeling the amount of laboratory testing can be reduced and designs can be optimized. Some parts of jet engines already have metallic parts that have been modeled on the microstructural level during the design phase. “If you have a good tool to predict mechanical response as a function of the microstructure you can avoid a lot of testing. For example, it is known that metals with small grains have better fatigue performance than those with large grains. If you introduce a new process that creates metals with large grains you can model the results before you physically test, reducing the amount of tests and optimizing the design,” says Segurado.
Additive manufacturing
In the USA, Illinois-based Questek Innovations is one of several companies involved in a US Air Force-sponsored challenge to help speed up the introduction of AM into aerospace manufacturing. Questek uses ICME principles to understand basic metallurgy and resolve materials issues for clients. Its Ferrium steels are being used in the latest helicopter transmission gears and for components on several aircraft, including for US Navy hook shanks on the T-45. The US Air Force challenge has asked companies to improve the accuracy of model predictions for metal AM, using Inconel nickel-chromium alloy 625 (IN625).
Jeff Grabowski, business development manager at QuesTek says, “Metallic AM is a process that metallurgists haven’t studied as thoroughly as casting or forging. There has been significant issues in simply taking legacy commodity alloy chemistries and using them in AM, such as cracking or poor performance.
“There are metallurgical challenges to resolve but also opportunities to take advantage of the rapid quenching of material to achieve microstructures not attainable via slower cooling manufacturing processes and therefore improve material performance.”
QuesTek’s engineers and scientists use a combination of computational materials engineering, which uses vetted databases of thermodynamic and kinetic properties of the elements and physics-based models to predict precipitation, grain boundary chemistry and microstructure of materials and the subsequent properties. They also use commercial Thermo-Calc software combined with their own propriety models. “We also do a lot of material prototyping, characterization and experiments to validate and calibrate our models,” says Grabowski. “We use scanning electronic microscopy, optical microscopy, XR Diffraction, hardness testing, mechanical testing. Atom Probe Tomography allows us to take a deep look at a materials structure to confirm presence of nanoscale precipitates and other features.”
Nanoscale metals
Xiaoling Zhou, postdoctoral research associate at Princeton University was co-author of a study published earlier this year at the University of Utah that has moved forward understanding of the structure of metals at the nanoscale level and could help create ultra-strong metals.
The study used samples of nickel procured from a commercial company and created inhouse with grain diameters as small as 3 nanometers. The samples were placed under intense pressures in a diamond anvil cell and the researchers used X-ray diffraction to observe what was happening at the nanoscale.
According to Zhou the method from the study can be used to measure the strength of other materials at the nanoscale. He says, “The high-pressure radial X-ray diffraction method through the diamond anvil cell enabled us to measure the strength of the nanometals in a way not available with conventional strength measurements such as tension tests or hardness measurements.”
According to the Hall-Petch relationship, metal strength increases as grain size decreases, down to around 10-15 nanometers. It was believed that below 10 nanometers the way grain surfaces interact with friction meant the grains would slide past each other under strain, leading to a significantly weaker metal.
But instead of a weakening, the tests showed that strength increased down to the smallest grain size available. The 3 nanometers sample withstood a force of 4.2 gigapascals before deforming irreversibly, 10 times stronger than nickel with a commercial-grade grain size. By applying pressure, the researchers were able to suppress the grain boundary sliding mechanism below 20 nanometers.
The results of the study, which were published in the journal Nature, could help to develop a new generation of ultra-strong metals by engineering their grain surfaces to suppress grain sliding. The metals would be suitable for any part of an air vehicle that requires high strength under extreme conditions. “Our results suggest a possible strategy for making ultra-strong metals,” Zhou says. “In the past, researchers believed the strongest grain size was around 10-15 nanometers, but we found we could make stronger metals at below 10 nanometers.
“The next step should be trying to make larger pieces of strong metals while confining the grain size to 3-10 nanometers. I’m not sure how long it will take to go for a commercial use but I’m optimistic it will happen.”
Zhou believes observing and engineering metals at the nanoscale level is the next step in metals research. “Since grains are very small and close to the atomic level, nanometals would exhibit distinct properties from micro-sized metals,” he says.
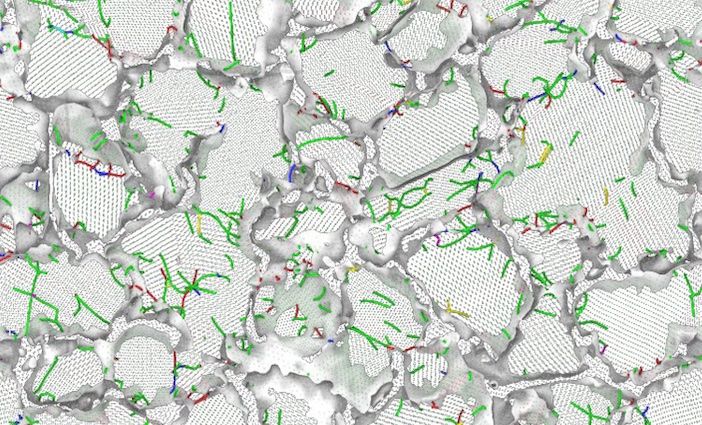
The aluminum, titanium, steel and refractory alloys used in aerospace applications are lightweight and strong with temperature resistance. Ceramic-based and polymer-based composites have issues with processability, reliable / consistent properties, geometric limitations and can be brittle or not have enough temperature resistance. Grabowski believes metals will therefore be used in aerospace applications for the foreseeable future and that ways of analyzing and testing these metals will continue to increase. “There is a lot of exploration into combining ICME with brute-force discovery tools like machine learning, artificial intelligence, neural networks and big data analysis. We have explored this and feel and have already demonstrated some potential and will continue to fine tune our capabilities here.”
However, the end of the journey for fully understanding metals on a microstructural level and less is some way off. “At the moment we are not even halfway to total understanding,” says Segurado. “You even have to do quantum mechanics to understand how atoms form their structure and interact with defects. But if you are less ambitious and want to just assess changes in the microstructure and design cleverer, we are around 80% from the end point.”
Model research
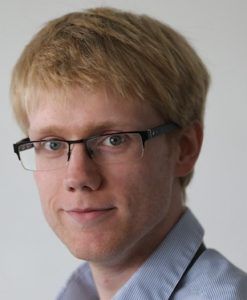
The four-year Airlift research program started last year at the UK-based Centre for Modelling and Simulation is developing simulation tools to model the development of microstructures in metals used in additive manufacturing (AM), to improve the finished part’s properties and performance so they can be used in aerospace applications.
The Centre for Modelling and Simulation (CFMS) is a not-for-profit research body with members companies from the aerospace, defence and automotive sectors. Alex Ballisat, senior research engineer and part of the model-based engineering team at CFMS says, “With AM instead of machining large forged fillets down, you are forming the microstructure as you make the part. We need process repeatability and reliability.
“Modelling is necessary because AM machines are expensive and not that common – you don’t have access to lots of them for testing.”
Researchers on the Airlift project are recreating the production environment of the AM machine as well as the material for its finite element modelling. The simulation models how the thermal mechanics of the process affects the creation of the microstructure over time, and predicts the mechanical properties of the final part.
Ballisat says, “It is a dynamic, time-dependent model that accounts for how fast the machine makes the part and how the duration of the process effects the part’s quality.
“The modelling process is a multi-objective optimization – we want to maximize the throughput but obtain a certain microstructure.”